Abstract
Hydrophobically associated (HA) hydrogels have attracted great concerns with their admirable properties, such as self-healing and shape memory. However, a few works have been devoted to apply HA hydrogels in practice, especially in wastewater treatment. This may be because of the non-ionic monomer composition and the poor mechanical properties after swelling. In this work, in order to improve the mechanical properties and absorption behavior of HA polyacrylamide (HPAM) hydrogel, hydrophobically associated polyacrylamide/cellulose nanofiber (HPAMF) composite hydrogels were prepared. It was found that by incorporating CNF (2 wt%), the tensile strength (≈0.276 MPa) was largely increased by 632% compared to HPAM hydrogels. The maximum Cu ion adsorption capacity of the HPAMF hydrogel (containing 2 wt% CNF) was 2.33 mmol g−1, about 86% over the HPAM hydrogel. The HPAMF hydrogels with self-healing, excellent mechanical and adsorption properties can be promisingly served as reliable absorbents, consequently satisfying the needs of practical application of heavy metal treatment.
Export citation and abstract BibTeX RIS

Original content from this work may be used under the terms of the Creative Commons Attribution 4.0 licence. Any further distribution of this work must maintain attribution to the author(s) and the title of the work, journal citation and DOI.
Introduction
Polymer hydrogels, with special three-dimensional network structure and soft-wet properties [1–5], show great prospects in numerous attractive fields such as water treatment, sensors, biomedical science, soft robots [6–14]. However, traditional polymer hydrogels always suffer from weak strength and unable to self-heal spontaneously, which are extremely important for practical applications [15–19]. Researchers are prompted to develop great hydrogels to meet these demands. Particularly, Hydrophobically associated (HA) hydrogel is a prospective one which is completely physically crosslinked. It possesses shape memory and autonomously self-healing properties [20–23]. And one-pot method is the most common and efficient way to fabricate HA hydrogels [24, 25]. In this way, some of the hydrophobic monomers and reactive agents will aggregate into hydrophobically micelles once assess to deionized water. Then some hydrophobic monomers will polymerize with hydrophilic monomers in the presence of radical initiators. Finally, hydrophobically micelles will associate with hydrophobic segments of polymer chains to come into being revisable physical crosslinkers. Most researches used to focus on regulating and controlling the alkyl chain content of hydrophobic monomers or changing the sort of surfactants to polish up the self-healing or mechanical properties of HA hydrogels [26–29]. While there are only a few works about enhancing comprehensive properties and exploring new functions of HA hydrogels for practical use. For example, HA hydrogels are merely used in wastewater treatment even though they have unique structures and many prominent superiorities. This may be because of the non-ionic monomer composition and the poor mechanical properties after swelling, which is resulted from their single physically cross-linked structure. Herein, it motivates us to fabricate a new type of HA hydrogels which process satisfying mechanical properties and considerable adsorption behavior for wastewater treatment. After taking all into consideration, we think that introducing nanofillers to fabricate composite HA hydrogels is the most facile and prospective way[24, 30]. Ran et al introduced graphene oxide(GO) into HPAM hydrogels to prepare composite hydrogels with ideally mechanical properties and applied them to dye absorption [24]. By introducing GO, the tensile strength of prepared composite hydrogels was largely improved, while the self-healing performance decreased sharply. This brings us a hint to find a better nanofiller for composite HA hydrogels.
Cellulose nanofiber (CNF) is a kind of nanofiber with high aspect ratio (diameter in 1–100 nm and length from several nanoscales to several microscales). Through the TEMPO-oxidized method and with the assistant of mechanical treatment, well dispersed nanofibers can be obtained [31, 32]. CNF owns a great deal of merits and is superior to other nanofillers in its light weight, high transparency, renewability and biodegradable properties. Its modulus and strength are close to that of inorganic fiber, showing extraordinary properties in physical and chemical aspects. As a result, CNF has been widely used to enhance composites [33–36] . More significantly, it is a good candidate to composite with polymer hydrogels and synergize their comprehensive performance [31, 32, 37]. The TEMPO-oxidized CNF with plenty of functional groups like carboxy and hydroxyl, is negatively charged [34, 38, 39] . So, CNF shows high affinity for heavy metal ions and may be a potential adsorbent for heavy metal ion treatment. However, it's hard to get rid of heavy metal ion from sewage by using CNF directly because it is hydrophilic and intractable to be separated from water [40–42]. As a result, integrating CNF with three-dimensional structural hydrogels to prepare heavy metal ion adsorbents seems to be more available. Up till now, a series of CNF/polymer hydrogels have been studied [43–46]. Because of the excellent properties of HA hydrogels, adding CNF into the HA hydrogels to prepare CNF / HA composite hydrogels seems to be an ingenious and reasonable way.
Herein, CNF was merged into the HPAM hydrogels to fabricate physically cross-linking HPAMF composite hydrogels. And they were used as adsorbents to remove Cu ion from sewage. Hydrogen bonds have formed between CNF and HPAM. And as the addition of CNF increasing, pore wall thickens and pore diameter decreases. Such structures have leaded to a great enhancement in mechanical properties. When suffered breaking, new physical crosslinkers would be formed in breaking part because of the hydrophobic association and hydrogen bonding. Furthermore, numerous functional groups on the surface of CNF can remarkably improve the adsorption capability of HPAMF composite hydrogels. In short, through compositing with CNF in a facial way, the HPAMF composite hydrogels with self-healing, excellent mechanical performance and adsorption behavior were prepared, which were well satisfied with the demands of application in heavy metal ion treatment.
Experimental
Materials
Stearyl methacrylate (SMA), sodium dodecyl benzene sulfonate (SDBS), potassium persulfate (KPS), Acrylamide were all provided by Kelong Chemical Reagent Factory (Chengdu, China). All these reagents mentioned above were used to prepare HA hydrogels and used as received. Microfibrillated cellulose (MFC) was obtained from Nanocyl S.A., Belgium and Daicel Chemical Industries, Ltd, Japan. Sodium hypochlorite (NaClO) solution, sodium bromide (NaBr) were also from Kelong Chemical Reagent Factory (Chengdu, China) and used as received. 2,2,6,6-Tetramethyl-1-piperidinyloxy (TEMPO, 98 wt%), purchased from Sigma-Aldrich, was used as an oxidant to obtain CNF. Anhydrous cupric sulfate (CuSO4), used to prepare Cu ion solution, was acquired from Kelong Chemical Reagent Factory (Chengdu, China). All the reagents were of analytical grade and the deionized water was used for all the preparations.
Preparation of cellulose nanofiber (CNF)
The method of preparing CNF was derived from the previous reported reference [31]. Briefly, 20 g MFC was added into 1 l deionized water and stirred overnight to obtain a dispersed solution, then 0.08 g TEMPO, 0.5 g NaBr were respectively dissolved into it. NaClO solution (200 mmol), working as an oxidant, was added into the solution to induce the oxidation reaction. Through adding 0.5 M NaOH solution, the PH value of the reaction system was controlled at the range of 10–10.5. To get tempo-oxidized cellulose suspension, the oxidation under continuous stirring at room temperature was in process and had been lasting for 6 h. For further vacuum filtrations and centrifugation at 10 000 rpm for 15 min, the CNF dispersion was finally obtained.
Preparation of HPAMF composite hydrogels
The HPAMF composite hydrogel was fabricated through soft in situ polymerization. Firstly, the hydrophobically monomer AM (20 wt %) and SMA (2.82 wt%) were simultaneously added into CNF dispersion, (CNF solid contents were 0.1 wt%, 0.5 wt%, 1 wt%, 1.5 wt%, 2 wt%), stirring for 30 min Then the surfactant SDS (6 wt%) was added into the mixed solution and let it stand still for 2 h at 34 °C. After SDS powders were completely dissolved, stirred continuously to form surfactant micelles in the mixed solution. Subsequently, the initiator KPS (1 wt% based on the total mass of AM and SMA) was dropwise added into the mixture to trigger the reaction. The mixture was injected into ampoules and then flame-sealed after being replenished with N2 for 10 min In the end, the ampoules filled with reactant solution were kept at 50 °C for polymerization to obtain HPAMF composite hydrogels. In this work, we used HPAMFn to simplify the spelling of composite hydrogels, where n represents for the relative content of the CNF to the mass of AM. For example, the prepared hydrogel was named HPAMF0.1 on account of that 0.1 wt% CNF was in the HPAM hydrogel. The HPAM hydrogels, used as control, was fabricated by the same way.
Characterization
The morphology of CNF and MCF was observed by scanning electron microscope (SEM, FEI). The hydrogels were quickly frozen by liquid nitrogen and freeze-dried into aerogels to characterize the porous structure by SEM. Fourier transform infrared spectroscopy (FTIR) spectra of the prepared hydrogels were recorded on a Nicolet 6700 (Thermal Scientific, USA) spectrometer.
Mechanical test
Tensile measurements were proceeded on the Instron 5576 universal test instrument with a 1 000 N load cell and tensile speed is about 100 mm min−1. The test specimens were cylindrical, whose diameter and length were 10 mm and 50 mm, respectively. To research the hysteresis properties of the prepared hydrogels, cyclic loading-unloading curves were tested. The maximum strain was at 1000% and crosshead speed of loading-unloading was 100 mm min−1. Dissipated energy was calculated by means of area under the stress-strain curve.
The Instron 5576 universal test instrument with a 1 KN load cell was also used for the compression test of cylindrical samples (20 mm in length ,10 mm in diameter). The crosshead speed was 2 mm min−1.
Swelling performance test
Swelling test was implemented by putting the specimens (5 mm in length, 10 mm in diameter) into deionized water for days at 25 °C. The swollen hydrogels were removed from the deionized water at set intervals, dried superficially with filter paper and then recorded the weight. By comparing the weight of swollen hydrogel to its initial weight, we can figure out the swelling ratio. The detailed equation is as follows:

where Wa is the weight of dried hydrogel in swelling test. While Wb represents the weight of swollen hydrogel.
Adsorption measurement
For absorption test, the prepared hydrogel in standard shape (30 mg) was immersed in Cu ion solution (20 mM) for 168 h. At different intervals, 2 ml liquid was removed from the solution to test its concentration C. The measurement was carried out in a digital water bath oscillator at 25 °C and 60 rpm. Before test, the pH of the solutions adjusted by NaOH (0.01 M) and HNO3 (0.01 M) was in the range of 5–5.5, which was tested by pH meter. The Cu ion concentration was measured by atomic absorption spectrophotometer (AAS, Z-8000, Hitachi, Tokyo, Japan). Absorption capability Q (mmol/g) at time t or at equilibrium is defined as the following equation:
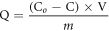
Where C0 and C (mmol/ L) represent the initial and absorbed concentration of Cu ion at time t or at equilibrium respectively. V(L) is the volume of solution and m (g) is the mass of the prepared hydrogel.
The reusability of HPAMFn hydrogel was tested by immersing 30 mg of the hydrogel in Cu ion solution (20 mM) for 24 h, then put the absorbed hydrogel into the 30 ml of 1 M HNO3 solution. It was placed in a in a digital water bath oscillator and shaken for 60 min at 60 rpm. In this process, Cu ion was unloaded by acid treatment. After that, the hydrogel was washed with deionized water for several times to remove residual Cu ion. The hydrogel was finally recovered and was employed for the next adsorption.
Results and discussion
Swelling performance of hydrogels
Microfibrillated cellulose (MCF), which derives from trees, straws or other plants, is thought to be an inexhaustible and environment friendly substance [47]. It's a raw material for the green dispersant of cellulose nanofiber (CNF). MCF suspension is obtained by dissolving MCF in water and then mechanically stirring. As shown in figure 1(a), it is purely white. Through the treatment of TEMPO-oxidation, the translucent CNF dispersion is prepared and shown in figure 1(b). In order to prove the well dispersibility of CNF, we have tested the microstructure of MCF and CNF by means of SEM as shown in figures 1(c), (d). CNF is well dispersed in solution because of the electrostatic interaction between carboxyl groups, which were generated by TEMPO-oxidation [31, 32, 37].
Figure 1. (a) Photograph of MCF dispersion. (b) Photograph of CNF dispersion. (c) SEM imagine of MCF dispersion. (d) SEM imagine of CNF dispersion.
Download figure:
Standard image High-resolution imageIn this work, CNF is introduced into the HPAM to prepare the HPAMF composite hydrogel. As shown in figures 2(ai)-(avi), the hydrogels are prepared into same size with10 mm in diameter and 4 mm in thickness. It's observed that the HPAM hydrogel is transparent. However, with increasing addition of CNF, HPAMF hydrogels whiten gradually, indicating network of CNF may be formed. These hydrogels are soaked in water to test swelling performance. And the swollen hydrogels about swelling for 168 h are exhibited in figures 2(a'i)-(a'vi). Contrasted to the shapes of original one, the HPAM hydrogel suffers largely swelling and much deformation. However, the hydrogels with more CNF are possessed of better dimensional stability so that it can maintain the shape in a better degree and only slight swelling is observed. The change of swelling ratio as function of swelling time is further shown in figure 2(b). The swelling ratio of HPAM hydrogel increases quite fast at the start and reaches up to 240% at 60 h. After that, it still keeps a slow trend of swelling and the swelling ratio is 328% at 168 h. While adding 0.1 wt % of CNF, the swelling rate of hydrogel gets much lower and the swelling ratio is 176% at 60 h, which is 26% less than that of HPAM hydrogel. In the following swelling process, the swelling ratio almost keeps constant. When the addition of CNF comes up to 0.5 wt % or more, the swelling rates decreases sharply and they all achieve swelling equilibrium within 48 h. The swelling ratio of HPAMF2 hydrogel is only 18% at 48 h and 16% at 60 h, which is decreased by 95% compared to that of HPAM hydrogel. This may attribute to the stronger internal network after incorporating with CNF, which has resulted in excellent stability of these hydrogels. What's more, as shown in figure 2(c), when the addition of CNF is more than 0.5%, swelling ratios about swelling for 168 h are always lower that of 60 h, which means the hydrogels are no longer swelling but deswelling in fact. The reason may be that the longtime swelling has resulted in the movement of polymer chains. And it has provided enough space for CNF to form stronger interaction.
Figure 2. Photographs of HPAM hydrogel (ai), HPAMFo.1 hydrogel (aii), HPAMF0.5 hydrogel (aiii), HPAMF1 hydrogel (aiv), HPAMF1.5 hydrogel (av), HPAMF2 hydrogel (avi) and swollen HPAM hydrogel (a'i), HPAMF0.1 hydrogel (a'ii), HPAMF0.5 hydrogel (a'iii) HPAMF1 hydrogel (a'iv) HPAMF1.5 hydrogel (a'v), HPAMF2 hydrogel (avi) about swelling in water for 168 h. (b) Swelling ratio of HPAM and HPAMF hydrogels. (c) Swelling ratio of HPAM and HPAMF hydrogels at 60 h and 168 h.
Download figure:
Standard image High-resolution imageMechanical and self-healing properties of HPAMF hydrogels
The mechanical performance is of critical importance to the application of hydrogels. Many investigations have focused on improving the mechanical properties of hydrophobically associated hydrogels [24, 30, 48, 49]. The stress-strain curves shown in the figure 3(a) are obtained through the tensile test, which quantitatively describe the tensile properties of these prepared hydrogels. HPAM hydrogel shows high malleability (3269%) but low tensile strength (0.041 MPa). After incorporating with 0.1 wt % CNF, the tensile strength (0.142 MPa) strikingly increases by 246% while the elongation at break only has a slight drop. It is suggested that by compositing with CNF, tensile property of the prepared hydrogel has been greatly improved with no significant decrease in ductility. As shown in figure 3(b), the tensile strength further increases with the increase of CNF. When the content of CNF is 2 wt %, tensile strength of the composite hydrogel reaches up to 0.276 MPa, which increases by 632% in contrast with HPAM hydrogel. This may be ascribed to the fact that CNF has formed hydrogen bonds with polyacrylamide chains and further formed another network of CNF with increasing additive amount. Therefore, internal structure is enhanced and become strong enough to bear greater tensile stress. What's more, such physically crosslinked structure can effectively transmit the force and partly dissipate energy as the bonds break and recombine. Furthermore, as shown in figure 3(c), the HPAMF hydrogels (ci), exhibited great mechanical properties visually when been crooked (cii), knotted (ciii) and Straightened while knotted (civ).
Figure 3. (a) Stress-strain curves of the prepared hydrogels. (b) Comparison of elastic modulus of the prepared hydrogels. (c) Photographs of illustrating the remarkable mechanical performance of HPAMF hydrogels: (ci) original, (cii) crooking, (ciii) knotting, (cⅳ) Straightening while knotted.
Download figure:
Standard image High-resolution imageCompression measurements can also reflect the mechanical performance of these hydrogels. The compression stress-strain curves are displayed in figure 4(a). The maximum value of compress strain is set at 90% and there is no fracture appearing in compression. All these compression stress have been sharply enhanced when the stain is 80%. Note that, if the content of CNF increases, the compress stress will heighten as well. The pure HPAM hydrogel possess the lowest compression stress of 0.329 MPa. While adding 2 wt% CNF into the composite hydrogel, the compress stress reaches up to 2.990 MPa. To visually represent the compress stress, it has been plotted into a histogram and shown in figure 4(b). Obviously, the compress stress has a rapid growth of 52% when the content of CNF turns to 2 wt% from 1.5 wt%. Figure 4(c) are photos on the process of compression, we can clearly see the strength difference between HPAM and HPAMF2 hydrogels. During the test, applying force is removed from these specimens at once the hydrogels are compressed into 10% of their original heights. The transparent specimen of HPAM hydrogel suffers large deformation and couldn't revert to the original state for a long time. This suggests that the single crosslinked HPAM hydrogels are too weak to against the applying force and the reversible hydrophobically associated interactions in the network recovered slowly. While compressed HPAMF2 hydrogel can recover to its original shape immediately once the applying force is removed. Because by introducing CNF into the prepared hydrogels, stronger hydrogen bonding network interaction has formed and outstanding network has been constructed. They won't deform even suffer huge compression stress, showing excellent compression-resilience property.
Figure 4. (a) Compress stress-strain curves of the prepared hydrogels. (b) Comparison of elastic modulus of the prepared hydrogels. (c) Photographs presenting the apparent degree of mechanical behaviors of the HPAM (ci-cv) and HPAMF2 (di- dv).
Download figure:
Standard image High-resolution imageTo quantify the anti-fatigue performance of hydrogels, the cyclic tensile was conducted. The loading-unloading curves are shown in figure 5. The maximum value of tensile strain is set at 1000% and the number of cycle times is 20. There are 10 min for recovery between each cycle. Every cycle forms a hysteresis loop. By calculating the area of the tensile hysteresis curves, the dissipation energies are obtained. The first load-unloading cycle of HPAM and HPAMF2 hydrogels are both the largest one compared with other hysteresis loops of their own, for the corresponding dissipated energy were 115.6 KJ m−13and 810.592 KJ m−13respectively. In later cycle tensile tests, the hysteresis loop of HPAM hydrogels shows small changes in areas and dissipated energy only has a slight drop. For example, the dissipated energy of the 2th and 20th cycle tensile test is 90. 779 KJ m−13and 68.807 KJ m−13, which have slightly declined of 22% and 40% compared with the 1th cycle tensile test respectively. The reason is that dissipated energies in cycles are mainly produced by breaking the reversible hydrophobical association bonds and they will recover over time after broken. While for HPAMF2 hydrogels, the loops become much smaller at the second cycle, illustrating a large decline has had in dissipated energy. The dissipated energy of the 2th cycle tensile test is 507.031 KJ m−13, which have declined by 37.4% compared to the 1th cycle tensile test. It may be attributed to the breaking interaction between CNF and polymer chains. In the next cycle tensile tests, the dissipated energy decreased slightly since the reformation of hydrophobic associated interactions. In general, the dissipated energies of HPAMF2 hydrogel are always larger than those of HPAM hydrogel during the loading-unloading cycle test, which attributed to the excellent toughness and fatigue resistance of HPAMF2 hydrogel.
Figure 5. (a) Cyclic stress-strain curves of HPAM hydrogel at a strain of 1000%. (b) Cyclic stress-strain curves of HPAMF2 hydrogel at a strain of 1000% strain. For each stress-strain cyclic test, the prepared hydrogels were provided 10 min for recovery. (c) Comparison of dissipated energies of HPAM and HPAMF2 hydrogel calculated from their stress-strain curves.
Download figure:
Standard image High-resolution imageNumerous methods have been applied to improve the mechanical properties of hydrogels. The way of introducing nanofillers is the most effective and successful one among those. But in this case, a detrimental effect on self-healing performance may generally be resulted in [7, 24, 30]. It's worth mentioning that in our work, CNF has led to massive increases in mechanical properties and with no damage to self-healing performance. In order to verify this, the self-healing and mechanical properties of HPAMF hydrogels were tested. As shown in the figure 6(ai), HPAM and HPAMF2 hydrogels are cut into uniform two pieces respectively, then piece them together and heal for 1 h under the condition of 25 °C and 60% humidity. After that, the cracks are invisible and the two pieces have completely recombined into wholeness again. As in figure 6(aii), the newly healed hydrogel has two different colors, the transparent one is HPAM hydrogel, which is in the left of the combination hydrogel. While the other half one in white is HPAMF hydrogel. The new healed hydrogel, have been stretched with large force and the process were shown in the figures 6(aiii)-(avi). With the stretch force increasing, the left half of HPAM hydrogel has a more and more severe deformation and ultimately cracked, while the HPAMF2 hydrogel is strong enough to stand the force and remain the same. And the healing interface does not break apart all the time. We can clearly see the good self-healing of hydrogels and notable mechanical strength differences between HPAM and HPAMF2 hydrogels. To compare the self-healing capability between HPAM and HPAMF2 hydrogels, self-healing efficiency test is conducted and calculated by using the ratio of the elongation at break of healed hydrogel to that of the initial hydrogel. As shown in figures 6(b), (c), after self-healing for 1 days and 3 days, the self-healing efficiencies of healed HPAMF2 hydrogel are 64% and 89%, matching 0.148 MPa and 0.238 MPa in tensile strength respectively. And the tensile strength of healed HPAM hydrogel is 0.033 MPa and 0.034 MPa, which corresponds to the higher self-healing efficiencies of 84% and 92% respectively. It is reasonable that the self-healing efficiencies of HPAMF2 hydrogel is not as good as HPAM hydrogel in a short time because the motion of segment is tougher after introducing nanofillers. However, the HPAMF2 hydrogel can recover to 95% through self-healing for half a month. It is noticeable that they can still recover to its original state as long as they are given enough time to self-heal. The self-healing of HPAMF hydrogels are almost attributed to hydrophobical association and hydrogen bonding interaction. Therefore, it can be concluded that, by incorporating with CNF, the mechanical performance of composite hydrogels will be enhanced greatly without damage of self-healing property.
Figure 6. Self-healing properties of the prepared hydrogels. (ai) The HPAM and HPAMF2 was cut into two parts respectively. (aii) Put the two parts together to self-heal for 1 h and then formed a new combination hydrogel without cracks. (aiii-avi) The new healed combination hydrogel can bear massive tension without breakage. (b) The stress-strain curves of the initial and the healed HPAM hydrogel. (c) The stress-strain curves of the initial and the healed HPAMF2 hydrogel.
Download figure:
Standard image High-resolution imageMechanism of reinforcement by adding CNF
Compared to traditional polyacrylamide hydrogel, HPAMF hydrogel is equipped with self-healing ability and better mechanical properties, which can be more widely used in various fields. The proof of excellent properties may be from its structure. The prepared hydrogels were prepared into aerogels through freeze-drying method so that the microstructures can be characterized by SEM in figure 7. The pore size distribution of HPAM hydrogel shown in figure 7(a) is heterogeneous, which is around 50 μm in diameter. And the pore wall is extremely thin and smooth. For such a poor structure, hydrophobic association hydrogel is not strong enough to against the permeation of water and maintain its shape when swelling. Therefore, HPAM hydrogel has a high equilibrium swelling ratio and is easy to be swollen and broken. As shown in figures 7(ai)-(avi), after been incorporating with CNF, fibrillar structure is observed. Moreover, pore wall thickens and pore diameter decreases with the CNF addition increasing.
Figure 7. SEM imagines of HPAM (ai), HPAMF0.1 (aii), HPAMF0.5 (aiii), HPAMF1 (aiv), HPAMF1.5 (av), HPAMF2 (avi). IR spectra of CNF, HPAM hydrogel and HPAMF hydrogel (b). Schematic imagine of the internal structure of HPAMFn hydrogel. (c).
Download figure:
Standard image High-resolution imageThe structures of CNF and hydrogels were further identified by IR spectra and the result is shown in figure 7(b). The IR peaks of HPAM hydrogel and HPAMF hydrogel are similar. However, new peaks of C–O (1058 cm−1) and C–O–C (940 cm−1) appear in HPAMF hydrogel, which are the characteristic groups of CNF. It's worth noting that -OH stretching vibration adsorption is shifted from 3423 cm−1 to 3337 cm−1 and C=O stretching vibration adsorption shifted from 1654 cm−1 to 1648 cm−1. Such an obvious redshift phenomenon can be attributed to the formation of hydrogen bonding between CNF and HPAM [30].
According to the discussions above, the enhancement mechanism can be clarified by the schematic descriptions shown in figure 7(c). HPAM hydrogels are a kind of conventional physical crosslinking hydrogels which crosslinked by hydrophobic association. Such a single crosslinked structure is not strong enough to be applied, though it possesses good self-healing ability. With the addition of CNF, another physical cross-linking is constructed by forming hydrogen bonding between functional groups of the CNF and HPAM. Furthermore, as the content of CNF increasing, networks of filaments have formed between the holes, leading to a large enhancement of mechanical properties. As for self-healing, when suffers from breaking, new physical crosslinkers will be constructed in breaking part because of the hydrophobic association and hydrogen bonding.
Adsorption behavior
Widening the applications of high-strength hydrogels is always the research emphasis. HA based hydrogels possess some superior properties that no other hydrogels have. However, vulnerably single crosslinked structure and lack of functions are barriers for them to application. Therefore, TEMPO-oxidized CNF is introduced into HPAM hydrogels to improve the mechanical properties and absorption behavior. To explore the absorption capacity and adsorption mechanism of HPAMF hydrogels, the Cu ion adsorption test was carried out. As shown in figure 8(a), the adsorption process can be divided into three stages: (1) In the initial 30 min, absorption rates of all hydrogels are high and all the Cu ion absorption capacities have reached above 90% of the maximum absorption capacity. (2) In 30–60 min, they keep a slow-growth in absorption capacity. (3) After 60 min, they almost stay at constant values. The maximum Cu ion absorption capacity of HPAM hydrogel is 1.25 mmol g−1. It is due to that the amide groups of hydrogels can complex with Cu ion. And the absorption capacity increases correspondingly with the CNF increasing and it reaches up to 2.33 mmol g−1 at 120 min when the addition of CNF is 2 wt%, which is 86% over the HPAM hydrogel. It may because that there are more negatively charged carboxyl groups to react with heavy metal ions.
Figure 8. (a) Effect of absorption time on the absorption capacity of the prepared hydrogels. Dots are the actual values of adsorption. Dotted lines are regression curves of pseudo-second-order model. (b) Schematic imagine of adsorption mechanism of the HPAMF hydrogel.
Download figure:
Standard image High-resolution imageTo figure out the adsorption mechanism, the absorption kinetics behaviors is simulated by the pseudo-first-order and pseudo-second-order models [50–53], and the expressions of pseudo-first-order models is descripted as follows:

Where qe and qt (mmol/g) are the corresponding amounts of adsorbed Cu ion at equilibrium and at time t. k1 is (g / mmol ·min) is the rate constant t of pseudo-first-order model, representing the Cu ion adsorption rate.
For pseudo-second-order mode, it can be generally expressed as follows:

Where k2 (g/mmol min) is the rate constant of pseudo-second-order model.
The calculated kinetics parameters of the pseudo-first-order and pseudo-second-order models are listed in table 1. It is found that the equilibrium absorption capacity qe calculated by pseudo-second-order model is more closely to the actual absorption capacity Qe and the correlation coefficients R2 are both bigger than 0.99. Moreover, the adsorption rate constant k of pseudo-second model increases as the addition of CNF increases. It is 0.04491 g (mmol ·min) for HPAM hydrogel and 0.10944 g (mmol ·min) for HPAMF hydrogel. So, it can be concluded that pseudo-second-order model is better fitted with the real absorption behavior, indicating that the absorption rate of HPAMF hydrogels is mainly controlled by chemical process.
Table 1. Adsorption kinetic parameters of pseudo-first-order model (a) and pseudo-second-order model (b) for adsorption of Cu ion.
pseudo-first-order model | pseudo-second-order model | |||||
---|---|---|---|---|---|---|
qmax (mmol/g) | k1(g/mmol min) | R2 | qmax(mmol/g) | k2(g/mmol min) | R2 | |
HPAM | 1.16894 | 0.9232 | 0.96831 | 1.28652 | 0.04491 | 0.99546 |
HPAMF0.1 | 1.15048 | 0.10765 | 0.97523 | 1.41635 | 0.07002 | 0.99742 |
HPAMF1 | 1.56478 | 0.09798 | 0.97489 | 1.7656 | 0.09829 | 0.99556 |
HPAMF2 | 2.26205 | 0.0898 | 0.98455 | 2.54733 | 0.10944 | 0.99463 |
The good adsorption capacity of HPAMF hydrogels may be attribute to complexation and electrostatic adsorption. As shown in figure 8(b), the amide groups on HPAM chain complex with Cu ion by sharing electrons. Moreover, because there are many negative carboxylate ions on the surface of CNF, the HPAMF hydrogel is negatively charged. So that it can form electrostatic adsorption with Cu ion via charge transfer. With more addition of CNF, the adsorption capacity of HPAMF hydrogels increases.
To measure the reusable property of the hydrogels, the desorption and absorption cycles was repeated for 4 times and the graphical illustration of the recycle process is shown in figure 9(a). Through adsorption, Cu ion has combined with carboxylate ion (–COO−) by means of electrostatic attraction. After acid treatment and washed with deionized water, some Cu ion has been removed and carboxyl groups have converted back into carboxylate ion. The detailed results of absorption-desorption recycle test is shown in figure 9(b). The adsorption rates of all the absorbents are very high but then decreases sharply after the first cycle. It may due to that there are lots of absorption sites on hydrogels at first, and heavy metal ions can facilely enter into the three-dimensional structure and be combine with active functional groups by way of complex reaction and electrostatic adsorption. However, though acid treatment, the active surface area of hydrogels decreased due to the shrinkage after acid treatment. In the further three recycle tests, the adsorption rates also have slightly drop, which can be attributed to the fact that carboxyl groups have lost electron partially by acid treatment and formed hydrogen bond with hydroxyl groups of CNF. After 4 cycles, the Cu ion absorption capabilities of HPAMF hydrogels are still higher than HPAM hydrogels and the values of the absorption capacity increase with the addition of CNF, showing that CNF is of great assistance for adsorption of HPAMF hydrogels.
Figure 9. (a) Photographs illustrating cycles of ion absorption and recycling absorbent using hydrochloric acid and deionized water. (b) Absorption capability of the prepared hydrogels for four absorption-desorption cycles.
Download figure:
Standard image High-resolution imageConclusion
In this work, CNF were introduced into HPAM hydrogels to prepare a new class of all physically cross-linking composite hydrogel. Compared with the HPAM hydrogel, the HPAMF hydrogel exhibited the higher strength, better compression-resilience and anti-fatigue properties. Besides, the self-healing property of the physical crosslinking HPAMF hydrogel was great as the HPAM hydrogel at the same condition. More importantly, the adsorption behavior of HPAMF hydrogel was sharply increased. The equilibrium adsorption capacity as well as cyclic adsorption capacity is increased in a large degree. In short, such an anti-fatigue, mechanical strong, self-healing and recyclable hydrogel possess high reliability in actual application in heavy metal ion absorption.
Acknowledgmnets
We would like to express our sincere thanks to the National Natural Science Foundation of China for financial support (Grant No. 51721091 and 51573102).
Notes
The authors declare no competing financial interest.