Abstract
Quantum dot solar cells seek to surpass the solar energy conversion efficiencies achieved by bulk semiconductors. This new field requires a broad selection of materials to achieve its full potential. The 12 nm spherical protein ferritin can be used as a template for uniform and controlled nanocrystal growth, and to then house the nanocrystals for use in solar energy conversion. In this study, precise band gaps of titanium, cobalt, and manganese oxyhydroxide nanocrystals within ferritin were measured, and a change in band gap due to quantum confinement effects was observed. The range of band gaps obtainable from these three types of nanocrystals is 2.19–2.29 eV, 1.93–2.15 eV, and 1.60–1.65 eV respectively. From these measured band gaps, theoretical efficiency limits for a multi-junction solar cell using these ferritin-enclosed nanocrystals are calculated and found to be 38.0% for unconcentrated sunlight and 44.9% for maximally concentrated sunlight. If a ferritin-based nanocrystal with a band gap similar to silicon can be found (i.e. 1.12 eV), the theoretical efficiency limits are raised to 51.3% and 63.1%, respectively. For a current matched cell, these latter efficiencies become 41.6% (with an operating voltage of 5.49 V), and 50.0% (with an operating voltage of 6.59 V), for unconcentrated and maximally concentrated sunlight respectively.
Export citation and abstract BibTeX RIS
1. Introduction
Increasing global energy demands, environmental concerns over carbon dioxide emissions, and finite fossil fuel reserves drive the transition to clean, renewable sources of energy. While solar power currently only fulfills a minute fraction of the world's energy needs, the International Energy Agency projects that it could meet up to one third of global demand by 2060 [1]. To meet this goal, advances will need to be made in photovoltaic (PV) efficiencies.
However, single junction PV cells are constrained by the Shockley–Queisser limit of 33.7% efficiency [2], which comes from the interplay between the solar spectrum and a semiconductor absorption model where photons with energy below the band gap are not absorbed whereas photons above the band gap are absorbed. Excess photon energy above the band gap is lost to phonon-related heating. The 33.7% maximum efficiency is obtainable with a material having the optimal band gap of 1.34 eV. Band gap energies lower than 1.34 eV (e.g. silicon at 1.12 eV) will be less efficient because too much of the photons' energy will be lost, while band gap energies higher than 1.34 eV will be less efficient because too many photons will go unabsorbed. The Shockley–Queisser limit can be surpassed with multi-junction PV cells, which have already reached efficiencies over 40% [3] and have a theoretical maximum of 86% [4]. These require multiple layers of materials with decreasing band gaps, breaking up the solar spectrum and allowing for less loss due to excess photon energy beyond the band gap of a given layer.
Quantum dot (QD) PV cells have shown promise for solar energy conversion [5], bringing a number of potential benefits unavailable to bulk semiconductors. QDs can potentially enhance multiple-excitation events—for example, multiple-exciton events have been shown to be at least twice as likely in PbSe QDs compared to the bulk material [6]—which further increases maximum efficiencies [7]. Furthermore, due to quantum confinement effects, the band gap of QDs can be tuned by controlling their size [8, 9]. This allows for greater flexibility in choosing appropriate band gaps for the different layers in a multi-junction PV device. Two and three junction QD PV cells have already been created and demonstrate increased conversion efficiency relative to any of their individual constituents [10].
Ferritin is a 12 nm diameter spherical protein with an 8 nm hollow interior, which naturally contains a ferrihydrite (Fe(O)OH) nanocrystal. Additional nanocrystals can be synthesized inside ferritin to replace the native ferrihydrite. Figure 1 shows representative TEM images of ferritin proteins with three different enclosed oxyhydroxide nanocrystals. Because ferritin is composed of 24 subunits, it contains a number of channels through which ions in solution can pass [11]. Using the right chemical processes, it is then possible to remove the ferrihydrite core and replace it with a diverse range of semiconductor nanocrystals [12–18]. The different ferritin subunits also provide added benefits, where one type (light chain) provides nucleation sites for self-assembling nanocrystals, while the other (heavy chain) contains the ferroxidase center which captures loose metal ions and reattaches them to the nanocrystal [11, 19]. Furthermore, by attaching charged molecules to its surface, ferritin can be deposited in ordered arrays on substrates [20–22].
Figure 1. Representative TEM images of ferritin protein (lighter shell) with synthesized encapsulated nanocrystals (darker core) of (a) Co(O)OH, (b) Mn(O)OH, and (c) Ti(O)OH.
Download figure:
Standard image High-resolution imageThe above benefits make the ferritin-nanocrystal complex a strong candidate for synthesizing a variety of light harvesting nanocrystals and organizing them for solar energy conversion. The ferroxidase center acts as a self-healing catalyst to prevent the effects of photo-corrosion and maintain nanocrystal integrity. A variety of possible nanocrystals inside the ferritin can provide many different band gaps, allowing for greater energy conversion efficiency. Traditional multi-junction solar cells made from bulk semiconductors require close lattice matching [23], limiting which materials can be used together. With nanocrystals in ferritin, this is not an issue as the protein itself can act as an interface between layers of different materials. Ferritin's electrical conductivity, and thus its ability to transmit the electric current generated by the enclosed nanocrystals, was shown to be relatively high through conductive atomic force microscopy [24]. This presents the potential for any type of material that can be synthesized within ferritin, regardless of crystalline structure, to be used in a multi-junction PV cell.
The architecture of a ferritin-based PV cell could mimic that of traditional QD solar cells or dye sensitized solar cells with the ferritin deposited onto a wide band gap electrode in an electrolyte solution to replenish electrons. Detailed schematics of such devices, including both current matched and independent circuit multi-junction devices, are readily available in the literature [25, 26].
While significant increases in efficiency have been obtained using bulk semiconductors in multi-junction PV cells [3], most of these devices use rare and/or toxic materials such as gallium, arsenide, or tellurium [27]. In this work we present data on iron, cobalt, manganese, and titanium oxyhydroxide nanocrystals inside ferritin, the latter three materials not being natively found. These metals are benign and abundant in the Earth's crust. Synthesis of these metal oxyhydroxide nanocrystals (M(O)OH where M is a metal) within ferritin is well established [11, 12, 14, 28], but their band gaps have not yet been well established. Specifically, the Ti(O)OH band gap has not been reported that we know of, and the Co(O)OH and Mn(O)OH band gaps have only been estimated via scanning tunneling spectroscopy (as 0.90 eV and 1.17 eV respectively) [29]; previously we have shown that STS measurements give less accurate results for band gaps than does optical absorption spectroscopy (OAS) [30]. Here we have used OAS to measure band gaps sensitively enough to see shifts in band gap from quantum confinement effects as particle size is changed; the results of the OAS measurements indicate the range of band gaps obtainable from titanium, cobalt, and manganese oxyhydroxide nanocrystals is 2.19–2.29 eV, 1.93–2.15 eV, and 1.60–1.65 eV respectively. Finally, we have used these band gap measurements to calculate theoretical efficiency limits for multi-junction PV cells using these materials. For concentrated sunlight efficiencies as large as 44.9% are theoretically obtainable, or 63.1% if another ferritin-based nanocrystal can be found with a band gap similar to silicon (i.e. 1.12 eV).
2. Materials and methods
Iron was removed from horse spleen ferritin (HoSF) (Sigma-Aldrich CAS# F4503) by dialysis with iron chelation, yielding apoferritin (empty protein shell) [31, 32]. Native HoSF was dialyzed against thioglycolic acid and 0.050 M TRIS. The resulting apoferritin solution was then purified by further dialyzing it against Milli-Q water with a 0.10% bicarbonate buffer at pH 7.4.
Co(O)OH cores were synthesized by following established methods [11] where Co(NO3)2 and H2O2 were periodically added to an apoferritin solution. The amount added was adjusted to target a range of core sizes at a rate of 100 metal atoms per addition per 10 min. The pH of the solution was maintained by addition of 10 mM NaOH.
Mn(O)OH cores were also synthesized by following established methods [12, 20]. A solution of MnCl2 was added to an AMPSO buffered solution of apoferritin to form the cores, resulting in a color change from clear to brown as the manganese oxidized and the core formed. The amount of MnCl2 added was varied between samples to target a range of core sizes at a rate of 100 metal atoms per addition per 10 min. The pH of the solution was also maintained by addition of 10 mM NaOH.
Ti(O)OH cores were made using the method from Klem et al [15]. Ti-citrate, ferritin, and buffer solutions were made to contain three times the concentration as found in the established method to give better amounts of absorption for the OAS measurements. A 150 W Xe arc lamp was used to illuminate the samples for titanium deposition, with a 1 cm water path to absorb IR light.
All samples were passed over a Sephadex G-75 column with pH 7.4 imidazole buffer to remove extraneous ions left over in solution. The actual sizes of the target cores for the various samples were determined by the Lowry method [33] for protein concentration and inductively coupled plasma mass spectrometry (ICP-MS) analysis for metal concentrations. Dividing the two concentrations gives the metal/ferritin ratio for each sample's cores.
Band gaps were measured in a dark environment using OAS as described in our previous work [21]. Broadband light from a 150 W Xe arc lamp was sent through a monochromator to select individual wavelengths. The light was sent through a mechanical chopper and then traveled through the ferritin solution and into a photodiode detector using standard lock-in techniques. The absorption coefficient α was then calculated by comparing the transmitted intensity to a control scan of an identical solution without the ferritin. Band gaps were determined by extrapolating linear fits from plots of α1/2 (for indirect gaps) and α2 (for direct gaps) versus photon energy. For indirect gap semiconductors, direct transitions are still possible at higher energies, so where measured we refer to the onset of such transitions as the 'direct gap' of these materials even though it is not the true band gap.
3. Experimental results
3.1. Apoferritin absorption
To distinguish between the absorption of the enclosed nanocrystals and the surrounding protein, we first measured the absorption profile of apoferritin (empty ferritin). We measured apoferritin to have a direct absorption edge at 4.0 eV, which was substantially higher than all of the absorption edges measured for the ferritin with nanocrystals. This gives us confidence in our band gap measurements for those nanocrystals. Additionally, this gives us an upper limit to the energies of photons which can reach the nanocrystals, so in our efficiency calculations below all incident photons with energy greater than or equal to 4.0 eV are assumed to be lost to the protein.
3.2. Co(O)OH band gap measurements
Co(O)OH nanocrystals were found to be indirect gap semiconductors with direct transitions possible at higher energies. A wide range of nanocrystal sizes was obtained, with the largest sample pushing the physical limit for the amount of atoms the ferritin can hold, approximately 4500 metal atoms with accompanying anions. Quantum confinement effects were seen in both direct and indirect gaps, though the direct gaps saw a more gradual change with core size. A steady band gap of 1.93 eV was reached with all of the larger cores, rising up to 2.15 eV in the small core case. Figure 2(a) shows the measured band gaps of various sizes of Co(O)OH nanocrystals. The disagreement with the previous measurement of 0.90 eV measured by STS [29] should be noted. Given that our envisioned applications are centered on light absorption, we take the band gap energy measured by optical absorption to be the relevant and more accurate value.
Figure 2. Band gap measurements of (a) Co(O)OH, (b) Mn(O)OH, and (c) Ti(O)OH nanocrystals plotted against core size. Squares show indirect gaps and circles show direct gaps.
Download figure:
Standard image High-resolution image3.3. Mn(O)OH band gap measurements
Mn(O)OH nanocrystals were also found to be indirect gap semiconductors with direct transitions possible at higher energies. The target range of Mn(O)OH core sizes resulted in two clusters of similar actual core size, as determined by ICP-MS analysis. Figure 2(b) depicts the band gaps measured in the Mn(O)OH samples, plotted as a function of core size expressed in terms of the number of metal ions. The smaller cluster of core sizes have band gaps of about 1.65 eV, 0.05 eV greater than their larger counterparts at about 1.60 eV, showing the clear effects of quantum confinement. These materials give an additional incremental step in covering more of the spectrum, absorbing lower energy light than either Fe(O)OH or Co(O)OH nanocrystals. We again note a disagreement with previous STS measurements of 1.17 eV [29], and for the same reasons take the band gap energy measured by optical absorption to be the relevant and more accurate value.
3.4. Ti(O)OH band gap measurements
Ti(O)OH nanocrystals were also found to be indirect gap semiconductors. We observe that through quantum confinement, we can tune the indirect band gap of Ti(O)OH ferritin samples over a range at least from 2.19 to 2.29 eV, giving added flexibility to selecting optimal band gaps in a multi-junction PV device. A direct transition (aka direct gap) appears to be around 3.8 eV, but due to the proximity to the absorption edge for apoferritin (4 eV) and an inability to decouple the two, we are not reporting precise values for the direct gaps.
4. Efficiency calculations—methods
We analyze the potential efficiency of solar cells using these ferritin-based nanocrystals in a manner similar to the historic calculation of Shockley and Queisser, which gives the efficiency limit of a single band gap solar cell as 33.7% (the 'Shockley–Queisser limit') [2]. We write the net current produced by a diode acting as a solar cell, I, in terms of a current source from absorbed photons creating electron-hole pairs and a current sink due to radiative recombination of some of the electrons and holes (non-radiative recombination is assumed to be small):

The photon current depends on the solar irradiance, which Shockley and Queisser calculated by modeling the Sun as a 6000 K blackbody; they also assumed that each photon with energy above the band gap produces exactly one electron-hole pair while photons with lower energy produce none. The recombination current is closely related to the well-known Shockley diode equation, i.e. the I–V curve of a standard diode. A plot of the net current vs. operating voltage V (such as we include below for our specific materials) shows that the maximum current is produced at V = 0, the current then decreases towards 0 as V is increased. Somewhere between those limits is the optimal operating voltage, obtained by maximizing the power, P = IV (i.e. by finding where dP/dV = 0). For silicon solar cells, with a band gap of 1.12 eV, the open circuit (zero current) voltage is 0.86 V, the optimal operating voltage is 0.77 V, and the maximum efficiency is 32.4%.
When multiple materials containing different band gaps are stacked on top of each other, the calculation becomes more complicated because the operating voltage must be optimized for each layer, but the optimal operating voltage of a given layer depends on the operating voltages of the surrounding layers, so a combined nonlinear optimization must be done. We generally follow the method of De Vos [4], although we have chosen to use the actual solar spectrum at the surface of the Earth (AM1.5 G [34]) instead of the 6000 K blackbody approximation. Our calculations proceed as follows, first the recombination current and then the photon current.
The recombination current arises from radiative recombination of some of the electrons and holes that takes place before they can escape the diode structure. In the dark and with no external bias, the number of electrons and holes per time lost to radiative recombination must equal the number of electrons and holes gained by blackbody radiation from its surroundings (due to the principle of detailed balance), which for the ith layer of the cell is given by:

where A is the top area of the cell (so that the total area above and below the layer is 2 A) and Eg,i is the band gap of the ith layer. N(f, T) is the blackbody radiation formula for photons of frequency f gained from the surroundings at temperature T, assumed to be 300 K, in units of photons s−1 m−2 Hz−1:

Note that our formula for N(f, T) is obtained from the usual blackbody formula, , in units of W Sr−1 m−2 Hz−1, by dividing by hf to convert watts to photons/sec, and integrating over angles in the context of a photon flux coming from the upper hemisphere, incident on a planar surface (which adds a factor of π).
As discussed by Shockley and Queisser [2], under normal operating conditions the increased number of carriers makes recombination much more likely. Specifically, the photon flux (photons per second) must depend on the product of n and p (concentrations of electrons and holes, respectively); this gives rise to an exponential dependence on operating voltage:

Or, written in terms of current (=qF), we have:

The photon current is given by a combination of the photons from the sunlight and photons from radiative recombination events from surrounding layers. The solar photon flux incident on the ith layer (i is measured from the top) which contributes towards current generation is given by

where is a geometrical factor explained below, S(λ) is the AM1.5 G solar spectrum (given in W m−2 nm−1), Ephoton(λ) is the energy of a photon of wavelength λ (=hc/λ). The limits of integration are as given because only photons of energy greater than Eg,i can create electron-hole pairs in the ith layer, and all photons of energy greater than Eg,i–1 have already been absorbed in the upper layers. The factor
accounts for the difference between unconcentrated, or normal sunlight, where the solar cell only sees a very limited solid angle of the Sun's radiation
, and concentrated sunlight, where the solar cell receives a higher density of photons
. Taking the extreme case, the maximal concentration is the amount of sunlight seen if the Sun were to fill the complete 2π solid angle of the sky
3
. (Obviously other assumptions, such as 300 K operating temperature, would break down under that concentration of photons.)
The photons impacting layer i due to radiative recombination events from layers i – 1 and i + 1 can be calculated using equations similar to equation (5), with the relevant area being A instead of 2A, and the limits of integration adjusted appropriately (all photons coming from layer i – 1 will be absorbed in layer i, but only those photons from layer i + 1 having energy above Eg,i will be absorbed in layer i):
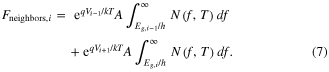
The equations for the top and bottom layers should obviously have the first and second terms removed, respectively.
Combining the two sources of photons and writing in terms of the current, we have the photon-generated current:
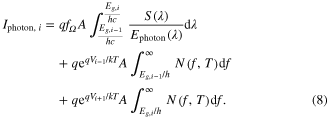
The optimal operating voltage for each layer is then obtained by maximizing the total electrical power across all n layers,

with respect to all of the Vi voltages. We then compare the total power produced using these operating voltages to the total incident power, found by integrating the entire solar power spectrum

The AM1.5 G spectrum ranges from 280–4000 nm due to lack of significant light outside of this region, so those values are used as practical limits of integration in these calculations.
Maximizing the electrical power yields a system of coupled nonlinear equations that is best solved numerically. Numerical solvers require good initial guesses of the operating voltages, especially as the complexity of the system increases with additional layers. Because the effects of mutual irradiance between layers are small compared to the light-induced current, solving an uncoupled system ignoring these factors gives an efficient starting point for numerical solving. Ignoring the coupling, the optimal operating voltage for the ith cell can be found by numerically solving the transcendental equation:

We also performed calculations for a current matched system, that is one in which the solar cells are arranged in series such that the current passes through all cells and the operating voltages add to give a larger total operating voltage. These calculations begin as previously described. The layer producing the lowest current is then identified and the voltages of the remaining layers are adjusted to give the same current as that layer. Changing the operating voltages impacts both the recombination current of the ith layer and the mutual irradiance seen by neighboring layers (equations (4) and (7)), so these values are then updated from the new operating voltages. Holding all other voltages constant, the optimal operating voltage of the current limiting layer is again solved for. This process is iterated until the voltages for all cells converge to fixed values and each layer produces the same current.
5. Efficiency calculations—results
We calculate the maximum efficiency from using Ti(O)OH, Fe(O)OH, Co(O)OH, and Mn(O)OH cores with indirect band gaps of 2.29, 2.14 [21], 1.93, and 1.60 eV respectively to be 38.0% for unconcentrated sunlight and 44.9% for maximally concentrated sunlight, obtained from operating voltages of 1.856, 1.680, 1.490, and 1.191 V for unconcentrated and 2.130, 1.954, 1.764, and 1.464 V for maximally concentrated sunlight. This maximum conversion efficiency is represented graphically in figure 3(a) where the heights of the color band represent the conversion efficiency of the solar cell at that wavelength. Figure 3(b) shows the I–V characteristics for each layer and their accompanying maximum power points. This 38% efficiency is greater than the Shockley–Queisser limit of 33.7% for any single junction solar cell, and far greater than the efficiencies obtainable by each type of nanocrystal individually (16.4%, 19.5%, 23.9%, and 29.9% respectively).
Figure 3. (a) Visual representation of the theoretical maximum efficiency for a ferritin-based multi-junction solar cell under unconcentrated sunlight found by maximizing equation (9). The black curve shows the AM1.5 G spectrum. Different colors (shades) represent different regions of the AM1.5 G spectrum absorbed by the different layers of material. Influence of a silicon-like bottom layer (labeled 'silicon substrate') on upper layers is small, so the colored (shaded) regions for the upper four layers are accurate for both including and excluding the silicon-like layer. From left to right the materials are Ti(O)OH, Fe(O)OH, Co(O)OH, Mn(O)OH, and silicon. (b) I–V Characteristic curves with colors (shades) corresponding to part a. Large dots show the maximum power points, indicating the operating voltages necessary to obtain maximum efficiency. The curves follow the reverse order from part a.
Download figure:
Standard image High-resolution imageWe also found the absorption by the ferritin shell to have trivial impact on the calculations due to the lack of significant UV light in the AM1.5 G solar spectrum, reducing efficiency limits by less than tenths of one percent. Furthermore, we do not consider the possibility for multiple excitation events, which have been shown more likely in quantum dots [6] and have the potential to further increase the maximum efficiency [7].
The main limiting factor in these efficiencies is the band gap of the bottom layer. A smaller final band gap gives rise to greater efficiency because fewer photons go unused. Ferritin has been successfully deposited onto a silicon substrate [35]; silicon with its 1.12 eV band gap could itself potentially serve as the bottom layer, capturing a large portion of the spectrum below the band gaps of all of these other metal oxyhydroxide nanocrystals. This is depicted in figure 3(a). An alternate possibility for increasing the efficiency would be to find a nanocrystal with a band gap similar to silicon that can be synthesized within ferritin. An excellent candidate for this is PbS; PbS nanocrystals have been synthesized inside of ferritin and photoluminescence experiments have shown a band gap of around 1.1 eV [18]. Be it through silicon or PbS (or another material with similar band gap), the efficiency limits in this case rise to 51.3% for unconcentrated and 63.1% for maximally concentrated sunlight.
The previous calculations have assumed that all absorbing layers are producing electrical energy independently. This would require each to drive a separate load, and the energy is made available at fairly low operating voltages. An alternate scheme is to connect the layers of the multi-junction cell in series. This allows for a single load and for a much higher operating voltage, but places additional constraints on the system. Namely, since the cells are connected in series and the same current passes through each cell, the layer producing the lowest current limits all the others. As an example of this, a recent materials system for a current-matched multi-junction PV cell has been proposed using III-V semiconductors; it allows up to 51.8% theoretical efficiency under 100 suns illumination (i.e. in our calculations) [36]. Using our selection of metal oxyhydroxide nanocrystals within ferritin, we produce a similar model for a current matched cell. As can be seen in figure 3(b), relatively little current is produced in the Fe(O)OH layer regardless of operating voltage; this is due to the proximity in band gap of native ferritin Fe(O)OH to Ti(O)OH (i.e. most of the light that would be absorbed by the Fe(O)OH layer has already been absorbed by the Ti(O)OH layer). Because this would substantially limit the overall current in a current-matched cell and negatively impact the efficiency, we have removed the Fe(O)OH layer from consideration in the current-matched case. Our current-matched calculations therefore include Ti(O)OH, Co(O)OH, Mn(O)OH and a 1.12 eV bottom layer. Band gaps were tuned through the region made possible by quantum confinement to give the optimal current-matched efficiency. The optimal band gaps four the four materials were calculated to be 2.29, 1.93, 1.64, and 1.12 eV, and the current-matched efficiencies are 41.6% for unconcentrated sunlight, 45.2% for 100 suns illumination, and 50.0% for maximally concentrated sunlight. The total operating voltages for the three types of illumination are 5.49, 5.96, and 6.59 V respectively. Figure 4(a) shows visually the efficiency of such a cell where the heights of the color band again represent the conversion efficiency of the solar cell at that wavelength. Figure 4(b) shows the I–V characteristics for the different layers, with points marking where each layer will operate. A summary of all efficiency calculation results is provided in table 1.
Figure 4. (a) Visual representation of the theoretical maximum efficiency for a ferritin-based current matched multi-junction solar cell under unconcentrated sunlight found by maximizing equation (9) under the constraint of all layers operating at the same current. The black curve shows the AM1.5 G spectrum. Different colors (shades) represent different regions of the AM1.5 G spectrum absorbed by the different layers of material. Influence of a silicon-like bottom layer (labeled 'silicon substrate') on upper layers is small, so the colored (shaded) regions for the upper four layers are accurate for both including and excluding the silicon-like layer. From left to right the materials are Ti(O)OH, Co(O)OH, Mn(O)OH, and silicon. (b) I–V Characteristic curves with colors (shades) corresponding to part (a). Large dots show the voltages at which each cell will operate. The curves follow the reverse order from part a.
Download figure:
Standard image High-resolution imageTable 1. Summary of the efficiency calculation results. The first three rows involve using only the ferritin-based metal oxyhydroxide nanoparticles whose band gaps we have measured. The next three rows involve using either silicon or nanoparticle with a silicon-like band gap (such as PbS) as an additional bottom layer. The next three rows similarly involve a silicon-like bottom layer, but in a current matching configuration in which the voltages combine.
fΩ (# Suns) | Operating voltages (V) | Theoretical efficiency (%) | |
---|---|---|---|
Ti, Fe, Co, Mn | 1 | 1.856,1.680,1.490,1.181 | 38.0 |
100 | 1.974,1.798,1.607,1.298 | 41.0 | |
Max | 2.130,1.954,1.764,1.453 | 44.9 | |
Ti, Fe, Co, Mn, Si | 1 | 1.856,1.680,1.490,1.191,0.748 | 51.3 |
100 | 1.974,1.798,1.608,1.308,0.864 | 56.4 | |
Max | 2.130,1.954,1.764,1.464,1.012 | 63.1 | |
Ti, Co, Mn, Si | 1 | 1.905,1.505,1.251,0.825 (5.49 total) | 41.6 |
(Current matched) | 100 | 2.024,1.622,1.370,0.944 (5.96 total) | 45.2 |
Max | 2.182,1.778,1.527,1.103 (6.59 total) | 50.0 |
6. Conclusion
We have precisely measured band gaps in three non-native metal oxyhydroxide nanocrystals synthesized within ferritin using OAS. The range of band gaps for materials within ferritin is extended from the 2.14 eV made possible by native Fe(O)OH up to 2.19–2.29 eV for Ti(O)OH and down to 1.60–1.65 eV for Mn(O)OH. The band gap for Co(O)OH lies in the middle at 1.93–2.15 eV. In each case the range listed is not due to experimental uncertainty, but rather the range made possible through size variation and quantum confinement effects. This spread of band gaps allows for efficient partitioning of the solar spectrum for increased solar energy harvesting efficiency. The theoretical maximum efficiency of a combined Fe(O)OH, Co(O)OH, Mn(O)OH, and Ti(O)OH nanocrystals within ferritin was calculated to be 38.0%, with unconcentrated sunlight and a multi-junction device, surpassing the single-material Shockley–Queisser limit on single materials. By concentrating the Sunlight incident on the cell, the maximal efficiency is further raised to 44.9%. Adding a ∼1.1 eV bottom layer (through a silicon substrate, or a PbS or similar ferritin-based nanocrystal) produces far greater efficiencies of 51.3% for unconcentrated and 63.1% for maximally concentrated sunlight. For a four material current-matched cell we calculated theoretical efficiencies of 41.6% and 50.0% for unconcentrated and maximally concentrated sunlight, with operating voltages of 5.49 and 6.59 V respectively. Future work will involve fabricating devices which can potentially realize some of these very high solar conversion efficiencies, and investigating lower band gap nanocrystals within ferritin.
Footnotes
- 3
Our symbol fΩ is closely related to the inverse of the fω symbol used by references [2] and [4], fω = 2.18×10 · 5 and fω = 1 for unconcentrated and maximally concentrated light, respectively; the difference is due to our use of the AM1.5 G spectrum (energy received by the Earth) rather than the blackbody spectrum of the Sun (energy leaving the Sun), and a more accurate value for the solid angle of the Sun.